Small Intestine Definition
The small intestine is the part of our gastrointestinal tract where most of our nutrient absorption takes place. Everything we eat and drink throughout the course of our day will make its way to the small intestine. The small intestine is commonly known as the “small bowel;” but despite its naming, it still spans an impressive twenty feet long with a circular diameter of about an inch. It is amazing to think that such a long intestinal tract is all encased within the relatively small space inside our abdomen. In terms of location, the small intestine will span from the pylorus (or, the muscular opening that connects the stomach to the opening of the small intestine) to the cecum (or the fecal pouch).
Upon closer inspection, the small intestine appears narrow and coiled. Its coiled nature, of course, helps the intestine fit within its limited allotted space within our bodies. Inside, the small intestine is covered by a soft lining that is contains many villi and microvilli. These little ridges or projections give the small intestine even more surface area from which to absorb nutrients. It is no surprise, then, that the small intestine is the principal site of our food’s molecular digestion. The small intestine is able to absorb these nutrients and shuttle them to the rest of the body via the bloodstream. Let us discuss the small intestine’s many functions in more detail.
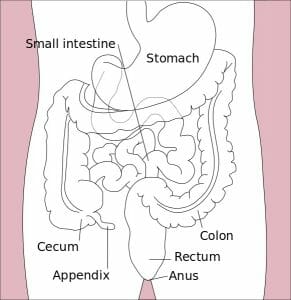
The image depicts an illustration of the organs of the alimentary canal.
Small Intestine Function
The small intestine is the site where up to ninety percent of our total nutrient and mineral absorption takes place. The remainder of the absorption is left to the stomach and the large intestine.
While the small intestine’s main function lies in absorbing nutrients from broken down food particles, it is important to note that the actual digestion of food undergoes two phases. Digestion begins with mechanical digestion in our mouths. By chewing and churning food with our teeth, the bonds that hold food particles together are physically broken down. This process is only carried forward by the starch-breaking action of amylase enzyme in our saliva. This digestion continues on in the stomach with the help of acids. This brings us to a second digestive phase, which is chemical digestion. Chemical digestion differs from mechanical digestion, in part because there are actual enzyme reactions taking place to break apart the molecular bonds that bind our food. This is made possibly with the help of bile acids that are released from the liver and the gall bladder. Likewise, chemical digestion relies on bile acids and enzymes that break the food down, and then give way to the release of minerals into our bloodstream and our body’s many tissues. Chemical digestion is a process that really only occurs in the small intestine, which is another fact that separates it from standard mechanical digestion that takes place at several points along the alimentary canal.
It is worth mentioning that the small intestine is a site that is very rich in enzyme activity. While some chemical activity does occur in the stomach with the help of acidic enzyme pepsin, chemical digestion continues, and thrives, in the small intestine. Even more distinctions are elucidated when we are tasked with investigating the digestion of the different macromolecules in our diets. In general, the main molecules that are absorbed by the small intestine include amino acids derived from proteins, fatty acids from lipids, and simple sugars derived from starches or complex carbohydrates, which we will discuss in more detail below. Furthermore, up to eighty percent of the water in our bodies is absorbed by the small intestine, as well as electrolytes like chloride, iron, potassium, and sodium ion. Ion channels will be crucial in replenishing and driving this life-sustaining process. Likewise, the small intestine has the important role of absorbing vitamins and minerals from our diet. Fat soluble vitamins K, A, D, and E are absorbed by simple diffusion along with dietary fats. Meanwhile, water soluble vitamins B and C will be absorbed by facilitated diffusion, as their hydrophilic nature precludes their simple entry into our cells. Vitamin B12, likewise, will be absorbed at the small intestine’s Ileum via active transport.
Digestion of Proteins
Proteolytic enzymes are those that target and break down peptide bonds within the proteins in our food. We, as a society, are quite familiar with what constitutes as protein in our diets; and in truth, a lot of protein is consumed throughout the world in the form of chicken, beef, tofu, and legumes. These enzymes will include trypsin and chymotrypsin, which are first released by the pancreas and will make their way to the small intestine to cleave proteins. Carboxypeptidase is an even more refined intestinal enzyme that is also released by the pancreas, but will split amino acids into singular amino acids. Protein digestion starts at the mouth and will continue, to a lesser extent, in the large intestine. Notably, amino acids are hydrophilic, or “water loving,” and will therefore require some help passing through the lipid barrier of our cells. They will generally follow primary active transport where an ATP molecule will be expended.
Digestion of Lipids
Lipases are likewise secreted by the pancreas and act on the fats in our diets. Lipases will break triglycerides into free fatty acids that can circulate within our bodies. But their action is further helped by bile salts that our liver and gallbladder secrete. Fatty triglycerides are very averse to the watery environments of our tissues. The bile salts act by enclosing the triglycerides within their structures until lipases can come and break them down. Transport of lipids and short-chained fatty acids will follow the rules of passive or simple diffusion through the hydrophobic lipid bilayers of our cells.
Digestion of Carbohydrates
The carbohydrates in our food will often consist of complex sugars. Their digestion into simpler sugars such as glucose is absolutely essential. Pancreatic amylase will help break down some of these carbohydrates, while the more tenacious fibers will experience bacterial breakdown in the large intestine. While fructose can be structurally absorbed by cells via facilitated diffusion, glucose will require secondary active transport.
Small Intestine Parts
The small intestine is further divided into three sections: the duodenum, the jejunum, and the ileum. The duodenum is the first and shortest section of the small intestine, which measures about fifteen inches long. It will receive chyme (or, a mix of partially digested food particles that is mixed with bile) from our stomachs. The duodenum’s intestinal cells will also secrete amylase, sucrase, and lipase enzymes that break down fats and sugars. The jejunum will follow suit, and is located near our belly buttons. The jejunum marks the end of our digestion of fats and carbohydrates. It is covered in villi and microvilli that make it the principal site of digestion. It is also a coiled structure that is thicker and has more blood vessels than the third and final section, the ileum. The ileum lies in our pelvic area, more or less, and is thinner and less vascular than the jejunum. The ileum’s main role is in absorption and it will absorb amino acids, lipids, fat soluble vitamins, and vitamin B12.
Quiz
1. Which method of transport do amino acids follow?
A. Passive diffusion
B. Simple diffusion
C. Primary active transport
D. Secondary active transport
Answer to Question #1
C is correct. Amino acids will be absorbed by cells via primary active transport, which requires the expenditure of one or more ATP energy molecules. Amino acids cannot be passively diffused through the lipid bilayer as they have a hydrophilic nature that makes it aversive to it.
2. Correctly label the first, middle, and third sections of the small intestine:
A. Ileum, duodenum, jejunum
B. Duodenum, ileum, jejunum
C. Jejunum, duodenum, ileum
D. Duodenum, jejunum, ileum
Answer to Question #2
D is correct. The first section begins with the duodenum, followed by the jejunum, and lastly the ileum. Digestion mainly takes place in the first two sections, with absorption taking place mostly in the ileum.
3. Correctly identify the main site of digestion, and the site of vitamin B12 absorption:
A. Duodenum; jejunum
B. Jejunum; ileum
C. Ileum; jejunum
D. Ileum: duodenum
Answer to Question #3
B is correct. The jejunum is very vascular and thick, making it the ideal site of digestion. The ileum, on the other hand, is the main site of absorption and importantly partakes in the absorption of fat soluble vitamins and vitamin B12!
References
- Hoffman, Matthew (2017). “Picture of the Intestines.” Web MD: Human Anatomy. Retrieved on 2017-08-30 from http://www.webmd.com/digestive-disorders/picture-of-the-intestines#1
- Mandal, Ananya MD (2017). “What does the small intestine do?” News Medical Life Sciences. Retrieved on 2017-09-01 from https://www.news-medical.net/health/What-Does-the-Small-Intestine-Do.aspx
- Schmidler, Cindy (2017). “Anatomy and Function of the Digestive System.” Health Pages. Retrieved on 2017-09-02 from https://www.healthpages.org/anatomy-function/anatomy-function-digestive-system/#Jejunum_Function
- Coleman, Ruth (2017). “What are the digestive enzymes that occur in each section of the small intestine.” Livestrong. Retrived on 2017-09-03 from http://www.livestrong.com/article/420133-what-are-the-digestive-enzymes-that-occur-in-each-section-of-the-small-intestine/
Small Intestine